Preparation and
characterization of series Gd1-xCaxCo1-xMnxO3
(0≤x≤1) compounds
Huo Guoyan, Dong Fuying, Yang Qing and Song
Dayong
(School of Chemistry and Environmental Science, Hebei University, Baoding 071002, China)
Received Oct. 16, 2006.
Abstract A series of
polycrystalline bulk samples of Gd1-xCaxCo1-xMnxO3
(0.0≤x≤1.0)
have been prepared. The structure, electrical transport and magnetic properties have been
studied by x-ray powder diffraction, electrical resistivity measurement and vibrating
sample magnetometer (VSM), respectively.
All composition Gd1-xCaxCo1-xMnxO3
(0.0≤x≤1.0) samples have a GdFeO3-type orthorhombic structure
(Pbnm space group). The lattice parameters a, b, c increase firstly and following decrease
with Ca and Mn doped. All the magnetic transition
temperatures of the samples are below 80.0 K. The electrical behaviors of all specimens
demonstrate insulator in the region of measured temperature and the electrical resistivity
decreases with Mn and Ca doped. The detailing analysis of electrical transport shows that
the electrical transport process of some samples are controlled by the transport of small
polaron model and that the electrical transport of the others can be described by
adiabatic small polaron hopping model at low temperature and by non-adiabatic hopping
model at high temperature, respectively.
Keywords Perovskite, Structure, Magnetism, Electrical transport
1. INTRODUCTION
With the discovery of colossal magnetoresistance (CMR) effect in manganites, hole-doping
perovskite manganites with unusual electronic transport and magnetic properties have
attracted considerable attention. These properties result from an intrinsic interaction
among charge, spin, orbital and lattice degrees of freedom which are strongly coupled to
each other[1-6]. Double exchange model combined with John-Teller effect was
used to explain part of these properties[7-9].
A great deal of work has been carried out on the manganites Ln1-xAxMnO3
since the discovery of colossal magnetoresistance properties in these compounds[10-11].
Also, many studies have been carried out on the Mn site doping effect (formula AB'1-xB''xO3)[12-15].
The research work reported by Gayathri et al.[16] suggests that the
substitution of Co for Mn dilutes the DE mechanism and changes the long range FM order of
La0.7Ca0.3MnO3 to a cluster glass-type FM order.
The electronic configuration of the Co3+ ions is more
complicated due to the possibility of the existence of the Co3+ ions in the low
spin, the intermediate spin and the high spin states. Most previous studies treat the
spin-state from the low-spin state to the thermally excited high-spin state [17],
since the high-spin (HS) state (HS, t42geg2)
is only 10-80 meV higher in energy than the low-spin (LS) state (LS,t62geg0)[18].
Therefore, it is easy to be excited to high spin state with temperature. Potze et al.[19]
reported that the interaction between high spin states of Co3+ is expected to
be antiferromagnetic. Thus trivalent Co is possible to be in a high spin state at room
temperature[20]. Based on our experimental results in our system we consider it
to be predominantly in the high spin state (HS, t42geg2).
To our knowledge, there is little data being available on coupled
A-B-site substitutions in ABO3[21]. On the basis of previously
published experimental data[21], we may expect that a coupled substitution
involving Ca and Mn would improve the properties of GdCoO3. So if Ca is doped
at Gd-site and Mn doped at Co-site, useful information for understanding the properties of
Gd1-xCaxCo1-xMnxO3 could be
obtained.
The purpose of the present study is to clarify the transport mechanism
in mixture of manganites Gd1-xCaxCo1-xMnxO3
(0≤x≤1) and to
show how the properties change with the replacement of Gd by Ca and Co by Mn.
2. EXPERIMENTAL
Polycrystalline samples of Gd1-xCaxCo1-xMnxO3
(0≤x≤1) were
prepared by standard solid-state reaction for x=0.0, 0.1, 0.3, 0.5, 0.7, 0.9, 1.0 from
stoichiometric amounts of Gd2O3, CaCO3, Co3O4
and MnO2, the purities of which were at least 99.9%. The Gd2O3
powders 99.9% dried for 5 hours at 8000C before use. The mixtures were ground
by hand in a mortar agate for at least 40 min and pressed into pellets, then preheated the
pellets for 8 hours at 8000C. Following, the mixtures were ground
once again. The ground powders were pressed into pellets and annealed at 11000C
in air for 24 h with intermediary grindings and finally cooled down the samples to 5000C
at 20C/min, then kept the samples in furnace till they reached room
temperature.
The crystal structure and the phase purity of the samples were examined
by X-ray diffraction (XRD) using Cu target at room temperature.
Temperature dependence of magnetization curve was measured by a
vibrating sample magnetometer (VSM) in a field of 0.5 T in the temperature range 80-300 K.
Electrical resistivity was determined by the standard four-probe DC method in the
temperature range 80-300 K.
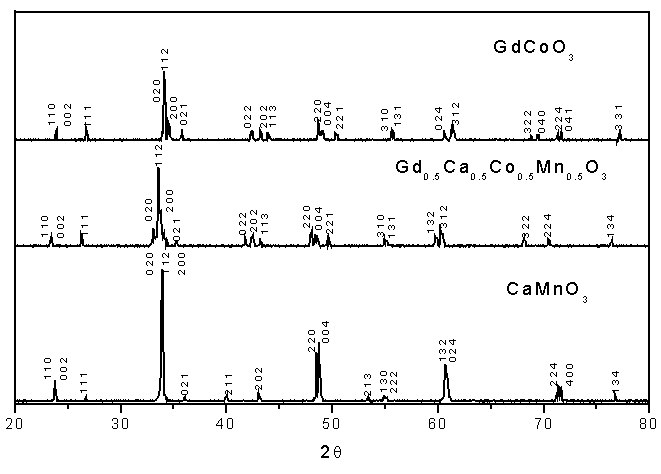
Fig. 1 Typical X-ray diffraction patterns of Gd1-xCaxCo1-xMnxO3
(0.0≤x≤1.0)
3. RESULTS AND DISCUSSION
3.1 Crystal structure
All compositions Gd1-xCaxCo1-xMnxO3
(0.0≤x≤1.0) of
synthesis by standard solid state technique were single-phase materials, which were
confirmed by powder XRD analysis (see Fig. 1). The XRD analysis results showed all samples
had a GdFeO3-type orthorhombic structure (Pbnm space group)[22].
This type of structure is common for rare earth perovskite-type oxides[23].
Each unit cell consists of four ABO3 units, and has the approximate dimensions ap× ap×2ap,
where ap is the lattice parameter of the ideal cubic unit cell. As regular
sites, a Ca2+ cation in a Gd3+ lattice position and a Mn4+
cation in a Co3+ position of the perovskite lattice. The determined lattice
parameters of the orthorhombic cell are shown in Table 1.
Table 1. Crystal structure, lattice parameters, unit cell volume, type of orthorhombic
structure and θCW (K) of the system Gd1-xCaxCo1-xMnxO3
(0.0≤x≤1.0).
x |
Crystal structure |
a(nm) |
b(nm) |
c(nm) |
v(nm3) |
a b c/
relation |
type of orthorhombic structure |
θCW (K) |
0.0
0.1
0.3
0.5
0.7
0.9
1.0 |
orthorhombic
orthorhombic
orthorhombic
orthorhombic
orthorhombic
orthorhombic
orthorhombic |
0.51978
0.52046
0.52700
0.53074
0.52836
0.52739
0.52636 |
0.53592
0.53860
0.53996
0.54152
0.53404
0.53038
0.52810 |
0.74087
0.74348
0.74579
0.74852
0.74559
0.74412
0.74384 |
0.206377
0.208412
0.212221
0.215129
0.210380
0.208143
0.206766 |
a<c/ <b
a<c/ <b
a<c/ <b
c/ <a<b
c/ <a<b
c/ <a<b
c/ <a<b |
O-type
O-type
O-type
O'-type
O'-type
O'-type
O'-type |
-266
-240
-125
-138
-148
-149
-180 |
In Table 2, the ionic
radii of the cations involved in this study are shown, according to shannon[24]. From
geometrical view the increase in radius on A site by substitution of Ca2+ for
Gd3+ is less than decrease in radius on B site by substitution of Mn4+
for Co3+ (see Table 2), so the lattice parameters should be decreased by the
coupled substitutions of A sites with that of B sites. In fact,the substitutions of Ca for
Gd and Mn for Co cause an increase of the lattice parameters for the series with
x=0.0-0.5, and a decrease of the lattice parameters can be observed for the series with
x=0.5-1.0 (see Table 1). The reason is described as following. In perovskite oxides, the
spin states of the d electrons in the Mn and Co ions form according to the strong Hund’s coupling. The vacant state with opposite spin lies higher in energy due to
exchange interactions. Both occupied and unoccupied spin states are furtherly split into
t2g and eg orbitals by the octahedral crystal field.
Increasing energy, these states are t2g , eg ,
t2g¯ and eg¯ . The electronic configurations
are for Co3+, for Co4+, for Mn3+ and for Mn4+, respectively. In a
solid, these states form bands. For Co3+ ions, the t2g and
eg bands are fully occupied, the t2g¯ is partly
occupied, and the eg¯ are empty. For Mn4+ ions, the t2g
bands are fully occupied, and the eg¯ are empty. The t2g¯
of Co3+ band, which is occupied by one electron, and the eg
of Mn4+ band, which can accommodate a maximum of two electrons per
ion, respectively, play a crucial role like the eg bands of Fe and
Mn in the La1-xCaxFe1-xMnxO3 system[25].
Because the Co4+ ion is easier to found than Fe4+ ion in
Co-containing perovskites[26], the system of Gd1-xCaxCo1-xMnxO3
(0.0≤x≤1.0) is
expected to have a similar band structure as that of La1-xCaxFe1-xMnxO3[25]
(see Fig. 2). The Co t2g¯ band overlaps with the Mn eg
band at a certain extent as shown in Fig. 2. In a mixed system of Co and Mn, the
widths and energies of their t2g¯ and eg bands
dictate the electron distribution of the Co and Mn ions. The energy diagram clearly shows
that t2g¯ electrons of Co3+ can hop to eg orbitals
of Mn4+ easily which can produce an amount of Co4+ and Mn3+
(see Fig. 2). Therefore, an increase of the lattice parameters for the series with
x=0.0-0.5 can be explained by change of oxidation state of Mn ion from +4 to +3 and Co ion
from +3 to +4 and ionic radius of Mn3+ bigger than that of Co3+ or
Co4+ (see Table 2). It also can be seen from Table 1 that in the Ca and Mn
dominant range with x = 0.5-1.0, the substitutions by coupling on A site and B site cause
the lattice parameters decrease. The reason is that doped Mn partly decreases to low
oxidation state, +3, based on the principle of neutrality and the Mn4+
concentration increases and the radius of Mn4+ is smaller than that of Mn3+
ion (see table 2). Therefore, the lattice parameters decrease with continuously doped Ca
and Mn ions with x = 0.5-1.0.
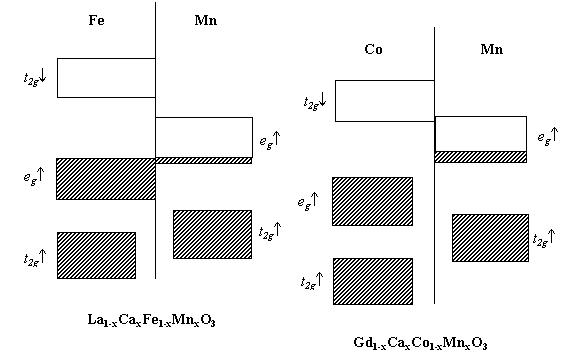
Fig. 2 Band structure of Fe and Mn in La1-xCaxFe1-xMnxO3
and Co and Mn in Gd1-xCaxCo1-xMnxO3
Table 2. Ionic radii of several
cations and their coordination-number (CN) in the perovskite lattice
Cations |
Ionic radius(nm) |
Gd3+(CN=9) |
0.1247 |
Ca2+(CN=9) |
0.132 |
Co3+(HS)(CN=6) |
0.075 |
Co4+(HS)(CN=6) |
0.067 |
Mn4+(CN=6) |
0.067 |
Mn3+(CN=6) |
0.0785 |
The
relation among the lattice parameters, a, b and c/ , can provide a measure of the distortion of the unit
cell[13]. This relation is showed in Table 1. Generally, two types of
orthorhombic structure are distinguished. The O-type orthorhombic structure, which is
characterized by the relationship a≤c/ ≤b, exists when the lattice deformation is
relatively small, while the O'-type orthorhombic structure, with c/ ≤a≤b, exists in the case of enhanced lattice deformation. The
structure of series Gd1-xCaxCo1-xMnxO3
compounds is O-type for 0.0≤x≤0.3 and O'-type for 0.5≤x<1.0,
respectively.
3.2 Magnetic properties
Fig. 3 presents the temperature-dependent magnetization (M-T) of the Gd1-xCaxCo1-xMnxO3
system under a magnetic field of 0.5 T (ZFC). It can be seen from Fig. 3 that the magnetic
transition temperatures of all the samples are below 80.0 K. The susceptibility presents
the Curie-Weiss-type behavior [χ = C/(T-θCW)] for the temperature above 80.0 K (see Fig. 4).
Where, C and θCW are Curie constant and
Curie-Weiss temperature, respectively. The values of θCW
of all samples are negative (see Table 1 and Fig. 4), indicating that the local
magnetization below magnetic transition temperature is associated with the
antiferromagnetic coupling. Curie-Weiss temperature is related to the strength of
antiferromagnetic interaction. Along with the increase of Ca and Mn contents from 0.0 to 0.3, the
Curie-Weiss temperatures increase to high temperatures gradually. This indicates
that antiferromagnetic interactions become slightly weak. And alone with
increasing Ca and Mn contents from 0.5 to 1.0, the Curie-Weiss temperatures slightly
decrease to low temperatures.
The observation of the variation of Curie-Weiss temperature along with
Ca2+ content (x) (see Table 1) may be due to that the double exchange
interaction between neighboring Co3+ or Mn3+ and Co4+ or
Mn4+ ions is relatively strong but short range which is responsible for the
formation of the ferromagnetic correlation and that the exchange interactions between Co3+
or Mn4+ and Co3+ or Mn4+ are strong and long range
antiferromagnetic. Therefore, the antiferromagnetic interaction is strengthened in the Co3+
dominant range or in the Mn4+ dominant range and accordingly the Curie-Weiss
temperature decreases to lower temperature. In a word, with Mn4+ substitution
for Co3+ up to Mn4+ content 0.3, Mn3+ concentration
increases, resulting in Curie-Weiss temperature fast moves to high
temperature, following with further increasing Mn4+ content, Mn3+
concentration decreases, resulting in Curie-Weiss temperature changes to slightly low
temperature.
Fig.
3 The temperature dependence of magnetization (M) of Gd1-xCaxCo1-xMnxO3
samples measured under H = 0.5T
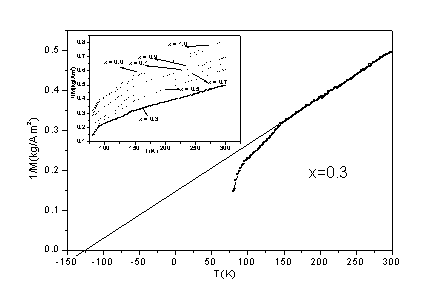
Fig. 4 The
reciprocal magnetization (1/M) for Gd1-xCaxCo1-xMnxO3
samples
3.3 Electrical resistivity
The temperature dependence of the electrical resistivity of the samples is shown in Fig.
5. Insulating behavior is observed for all samples in the studied temperature range. No I–M transition in the series Gd1-xCaxCo1-xMnxO3
(0.0≤x≤1.0) is
observed in the studied temperature range. The electrical resistivity of the Gd1-xCaxCo1-xMnxO3
(0.0≤x≤1.0)
solid solution slowly decreases with increasing x when the temperature is above about 100K. As some
samples’ electrical resistivity is too high to
measure on our apparatus at low temperatures, we can not obtain the values of electrical
resistivities below 100 K. It can be seen from Fig. 5 and the inset of Fig. 5 that the
electrical resistivity of GdCoO3 compound is the biggest in the series Gd1-xCaxCo1-xMnxO3
(0.0≤x≤1.0)
and of all other samples decrease with Ca and Mn doped (except x = 0.3 at low temperature
(below 157K)). The electrical resistivity is more than 2 order of magnitude higher at room
temperature for x = 0.0 (GdCoO3) than x = 1.0 (CaMnO3).
In order to get more insight into the electrical transport process, the
electrical resistivity data were fitted to various electrical transport models. Fig. 6 shows
the electrical transport behaviors for x =0.1, 0.3, 0.5, 0.7 and 1.0 samples. It can be
seen from Fig. 6 that two steps transport of small polaron model is confirmed for x = 0.1,
0.3 and 0.5 (see Fig. 6a) and that adiabatic polaron is demonstrated for x = 0.7 and 1.0 (see
Fig. 6b). For the specimens of x = 0.0 and 0.9, the electrical transport behaviors shows
adiabatic polaron at low temperature and non-adiabatic polaron at high temperature,
respectively.
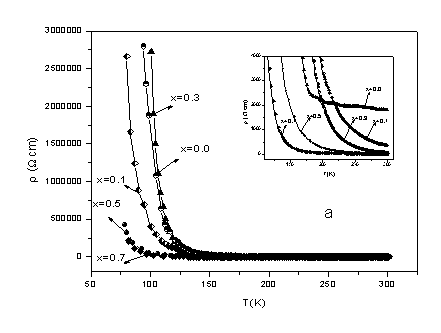
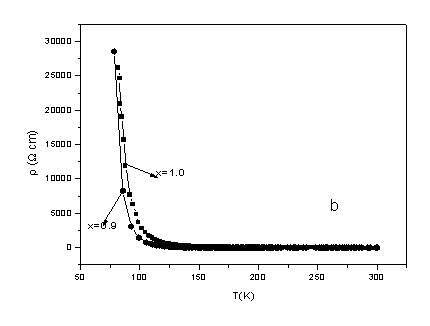
Fig. 5 The temperature dependence of electrical resistivity for polycrystalline
samples of the solid solution Gd1-xCa x Co1-x MnxO3
(0.0≤x≤1.0) as
a function of temperature
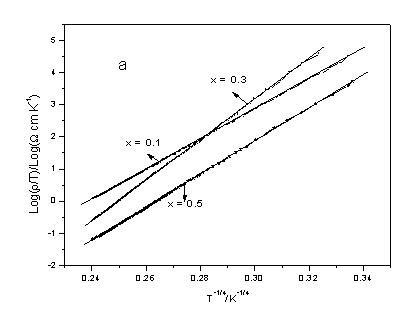
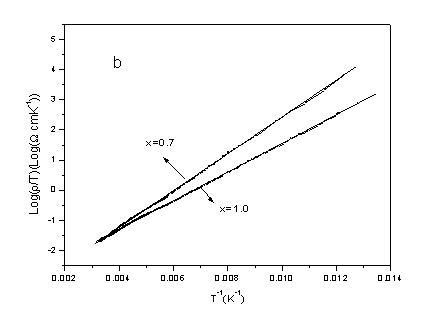
Fig. 6 (a) log r / T vs. T-1/4, (b) log r / T vs. T-1 plots
for selected samples
4. CONCLUSION
We have investigated the structure, electrical transport and magnetic properties of
the series Gd1-xCaxCo1-xMnxO3 (0.0≤x≤1.0) compounds. The lattice
parameters a, b, c increase firstly with x≤0.5 and
following decrease with x≥0.5. The magnetic
transition temperatures of all the samples are below 80.0 K. The values of θCW of all samples are negative. The electrical behaviors
of all specimens demonstrate insulator and the electrical resistivity decreases with Mn
and Ca ionic doped. The detailing analysis of electrical transport shows that the
electrical transport process of some samples are controlled by two steps transport of
small polaron model, some by adiabatic small polaron hopping model in the whole studied
temperature range and others of the electrical transport can be described by adiabatic
small polaron hopping model at low temperature and by non-adiabatic hopping model at high
temperature, respectively.
ACKNOWLEDGEMENT This work was
supported by Foundation for Doctor, Education Department of Hebei Province (B2002102), P.
R. China.
REFERENCES
[1] Helmolt R V, Wecker J, Holzapfel B, et al., Phys. Rev. Lett., 1993, 71: 2331-2333.
[2] Jin S, Tiefel T H, McCormack M, et al., Science, 1994, 264: 413-415.
[3] Gong G Q, Canedy C, Xiao G, et al., Appl. Phys. Lett., 1995, 67: 1783-1785.
[4] Xiong G C, Li Q, Ju H L, et al., Appl. Phys. Lett., 1995, 66: 1427-1429.
[5] Asamitsu A, Moritomo Y, Tomioka Y, et al., Nature, 1995, 373: 407-409.
[6] Caignaert V, Maignan A, Raveau B, Solid State Commun., 1995, 95: 357-359.
[7] Millis A J, Littlewood P B, Shraiman B I, Phys. Rev. Lett., 1995, 74: 5144-5147.
[8] Millis A J, Shraiman B I, Mueller R, Phys. Rev. Lett., 1996, 77: 175-178.
[9] Millis A J, Phys. Rev. B, 1996, 53: 8434-8441.
[10] Kusters R M, Singleton J, Keon D A, et al., Physica B, 1989, 155: 362.
[11] Chahara K, Ohno T, Kasai M, et al., Appl. Phys. Lett., 1993, 63: 1990.
[12] Schuddinck W, Tendeloo G V, Barnabe A, et al., J. Magn. Magn. Mater., 2000, 211:
105-110.
[13] Kostogloudis G Ch, Fertis P and Ftikos Ch, Solid State Ionics,
1999, 118: 241-249.
[14] Pi L, Zheng L and Zhang Y, Phys. Rev. B, 2000, 61: 8917-8921.
[15] Xiong C M, Sun J R, Li R W, et al., J. Appl. Phys., 2004, 95: 1336-1342.
[16] Gayathri N, Raychaudhuri A K, Tiwary S K, et al., Phys. Rev. B,
1997, 56: 1345-1353.
[17] raccah p m and goodenough j b, phys. rev., 1967, 155: 932.
[18] asai k, gehring p, chou h, et al., phys. rev. b, 1989, 40: 10982.
[19] Potze R H, Sawatsky G A, and Abbate M, Phys. Rev. B, 1995, 51: 11501.
[20] Asthana S, Nigam A K and Bahadur D, J. Appl. Phys., 2005, 97: 10C101-3.
[21] mitchell r h, chakhmouradian a r, j. solid state chem., 1999, 144: 81-85.
[22] Geller S, J. Chem. Phys., 1956, 24: 1236.
[23] Demazeau G, Pouchard M and Hagenmuller P, J. Solid State Chem., 1974, 9: 202-209.
[24] Shannon R D, Acta Crystallogr., 1976, A32: 751-767.
[25] Banks E, Tashima N, J. Appl. Phys., 1970, 41: 1186-1187.
[26] Carvalho M D, Ferreira L P, Colomer M T, et al., Solid State Sciences, 2006, 8:
444-449.
钙钛矿型复合氧化物Gd1-xCaxCo1-xMnxO3
(0.0≤x≤1.0)的制备与表征
霍国燕 董福营 杨青 宋大勇
(河北大学化学与环境科学学院 河北 保定 071002)
摘要 利用固相烧结法制备了Gd1-xCaxCo1-xMnxO3
(0.0≤x≤1.0),分别利用X射线粉末衍射仪及振动样品磁强计对它们的结构、电学性质和磁学性质进行了研究。在整个掺杂范围内,样品的相结构都为GdFeO3型结构(即Pbnm空间群)。晶胞参数a、b、c随着Ca和Mn掺入量的增加先增大后减小。所有样品的磁转变温度都低于80K,在所测的温度范围内都呈现绝缘体性质。而且样品的电阻率随着Ca和Mn的掺入量的增多而相应地降低。另外还对样品的导电机制进行了研究,分别对样品进行了各种电输运模型的拟合,发现有的样品的导电机制符合小极化子模型,有的则在低温时符合绝热小极化子跃迁模型,而在高温时却符合非绝热变程跃迁模型。
关键词 钙钛矿,结构,磁性,电运输,磁阻
|